Atomic Theory II: Ions, neutrons, isotopes and quantum theory
Listen to this reading
Did you know that energy is not released in a continuous flow, but rather is released in “packets”? This discovery, known as quantum theory, changed the way we understand the basic properties of the atom. Many other advances in atomic theory were made in the 20th century, including the discovery of the neutron, which made the atom bomb possible.
The earliest ideas about matter at the atomic level were built over many centuries. Starting with the ancient Greeks, and moving through to the beginning of the 19th century, the story unfolds relatively slowly. (You can read more about this is in our modules Early Ideas about Matter: From Democritus to Dalton and Atomic Theory I: The Early Days.) Despite the slow pace, it is crucial to understand that the process was a methodical one as each scientist built upon earlier ideas. This gradual, logical progression, where atomic structure evolved from being a simple, philosophical idea, through to the ultra-sophisticated world of the Higgs boson particle discovered in the early part of the 21st century, represents a wonderful example of the evolution of a scientific idea, and the application of the scientific process. In fact, one could argue that the history, struggle, and achievement that is threaded through the development of understanding matter at the atomic level is the quintessential story of the scientific method.
Evidence that led to change
The story of atomic theory first encounters reproducible, scientific (evidence based) proof in the late 18th century. French chemists Antoine Lavoisier and Joseph Proust, with their Law of Conservation of Mass in 1789 and Law of Definite Proportions in 1799, respectively, each laid the groundwork for Englishman John Dalton’s work on the Law of Multiple Proportions (Dalton, 1803). Given that many centuries had elapsed between the earliest ideas of the atom and Dalton’s work, it would be fair to say that the evolution of atomic theory had been a gradual one, with progression in the field being steady rather than spectacular. But that was all about to change, and quite dramatically.
The most intense period of progress took place between the late 19th and early 20th century, and it hinged heavily on the work of a Danish physicist named Niels Bohr. Like so many before him, Bohr built upon the work of his predecessors, and for Bohr, part of that foundation had been built by Ernest Rutherford.
Based upon a series of experiments, Rutherford proposed the planetary model of the atom in which electrons swirled around a hard, dense nucleus (see Atomic Theory I: The Early Days). While Rutherford’s model explained many observations accurately, it was found to have flaws.
Bohr advances Rutherford’s ideas
Rutherford’s planetary model of the atom was based upon classical physics – a system that deals with physical particles, force, and momentum. Unfortunately, this same system predicted that electrons orbiting in the manner that Rutherford described would lose energy, give off radiation, and ultimately crash into the nucleus and destroy the atom. However, for the most part, atoms are stable, lasting literally billions of years. Furthermore, the radiation predicted by the Rutherford model would have been a continuous spectrum of every color – in essence white light that when passed through a prism would display all of the colors of the rainbow (Figure 1).
But when pure gases of different elements are excited by electricity, as they would have been when placed in the newly discovered electric-discharge tube, they emit radiation at distinct frequencies. In other words, different elements do not emit white light, they emit light of different colors, and when that light is passed through a prism it does not produce a continuous rainbow of colors, but a pattern of colored lines, now referred to as line spectra (Figure 2). Clearly, Rutherford’s model did not fit with all of the observations, and Bohr made it his business to address these inconsistencies.
Figure 2: The visible light spectrum is displayed at the top and line spectra for three elements - hydrogen, neon, and iron - are below.
image ©Neon spectrum: Deo FaventeIn 1911, Niels Bohr (Figure 3) had just completed his doctorate in physics at the University of Copenhagen and was invited to continue his work at the University of Manchester in England by Rutherford. Rutherford had already significantly advanced atomic theory with his groundbreaking gold foil experiment, but Bohr’s genius was in taking the Rutherford model and advancing it further.
Figure 3: Niels Bohr
It wasn’t Bohr who had come up with the original idea of a planetary model of the atom, but he was the one who took the fundamental concept and applied new ideas about quantum theory to it. This leap was necessary to explain the new evidence that challenged the old model, and to subsequently formulate a new, ‘better’ model.
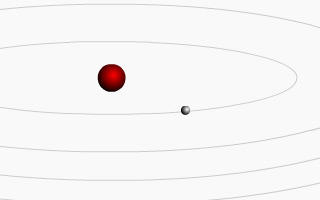
Interactive Animation: Bohr’s Atom
Comprehension Checkpoint
Max Planck and Quantum Theory
It’s easy to think of light, and other forms of energy, as continuous. Turn up the dimmer switch on your lamp, and the lamp gets gradually brighter. However, by the late 1800s, physicists were beginning to suspect that this was not in fact true. Classical physics models failed to accurately predict black-body radiation; in other words, classical physics did not accurately predict the energy given off by an object when it was heated.
The German physicist Max Planck solved this problem in 1903 by proposing that black-body radiation energy had to be quantized, i.e., that it could only be released or absorbed in specific ‘packets’ that were associated with specific frequencies. This solved the black-body problem and was consistent with the observed experimental data. Thus, quantum mechanics was born.
Despite the advances that he and others made using this idea, interestingly, Planck remained quite skeptical of quantized energy for many years. He insisted that the calculations that he had done, and the conclusions that he had reached, were somehow a sophisticated mathematical trick and that ultimately the old, classical model would prevail. After all, it had been around for approximately 200 years and had stood up to some pretty intense scrutiny.
Quantum Theory advances
In 1905, Albert Einstein published a series of papers proposing that light also exhibited quantum behavior (Einstein, 1905). Sometimes described as Einstein’s Annus mirabilis (miracle year), the papers taken together, and combined with Planck’s work, allowed Bohr to marry the nature of the atom with physics to usher in a new dawn of understanding in atomic theory.
In 1913, building on Planck’s and Einstein’s theories of quantization, Bohr proposed that the electron itself was quantized – that it could not exist just anywhere around an atom (as suggested by the Rutherford model) but instead could only be found in specific positions, with specific energies. The electron could transition to different positions, but only in discrete, defined steps. It could not spin in any location around the nucleus of an atom but instead was restricted to specific areas of space – much like the planets in our solar system are restricted to specific paths.
Being negatively charged, electrons are attracted to the positive protons in the nucleus of an atom, and will normally occupy the orbital, or path, within an atom that is closest to the nucleus if it is available. This state, which has low potential energy, is called the ground state. By exposing the electrons to an external source of energy such as an electric discharge, it is possible to promote the electrons from their ground state to other positions that have higher potential energies, called excited states. These ‘excited’ electrons quickly return to lower energy positions (in order to regain the stability associated with lower energies), and in doing so they release energy in specific frequencies that correspond to the energy differences between electron orbitals, or shells (see quantum behavior simulation). Bohr’s mathematical equations further predicted that electrons would not crash into the nucleus in a manner that classical physics – and Rutherford’s model – had predicted. This was another crucial realization that made a jump from one paradigm (classical physics) to a new one (quantum physics).
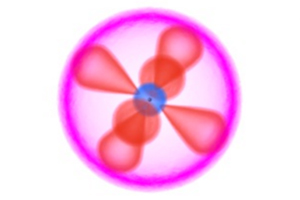
Interactive Animation: Atomic and ionic structure of the first 12 elements
Bohr’s discovery that Planck’s quantum theory could be applied to the classical Rutherford model of the atom, and could account for the observed shortcomings in the original model, is another beautiful example of how scientific theory uses prior evidence, coupled with new experimental observations, to adapt, develop, and change models and understanding over time. Science is usually advanced by contemporary scientists building on the work of predecessors and, as Isaac Newton put it in his 1676 letter to Robert Hooke (both prominent scientists of their time), by their “standing on the shoulders of giants.” Bohr’s work built on the theories of those before him, and extended them to explain the experimentally observed line spectra of atoms in a mathematical proof that made perfect sense.
Comprehension Checkpoint
The formation of ions
While Bohr’s work seemed to explain the curious phenomenon of line spectra, a number of lines had been observed in the spectra of hydrogen that did not fit Bohr’s theory. At first glance this appeared to poke holes in Bohr’s ideas, but Bohr was quick to offer an explanation. He suggested that the lines in the spectrum for hydrogen that could not be accounted for were actually caused not by hydrogen atoms, but rather by an entirely different species altogether. So, what were these different species, and how did they come to be?
Almost 30 years prior to Bohr's publishing his famous trilogy of papers in the Philosophical Magazine and Journal of Science in 1913, the idea of particles having the ability to carry some kind of charge had been established by the Swedish scientist Svante Arrhenius and the Englishman Michael Faraday. These charged particles had been christened ions.
Atoms are electrically neutral, meaning that the number of positive protons in any given atom equals the number of negative electrons in the same. Since the positive charge is exactly cancelled by the negative, the atom has no overall electrical charge. However, just as it is possible to excite an electron to a higher orbital, as Bohr’s work had shown, it is also possible to give an electron sufficient energy to overcome the attraction of the nucleus completely and to remove it from the atom entirely. This has the effect of unbalancing the electrical charge and results in the formation of a species with an overall positive charge – called a cation. For example, a sodium atom can lose an electron to form a positively charged sodium cation (Equation 1); the energy associated with the ejection of the first electron from any atom is called the first ionization energy.
Cations that are formed by the ejection of one electron can be further ionized by losing additional electrons, and in the process forming another ion, this time with a 2+ charge. The energy required for the ejection of the second electron is known as the second ionization energy. Although it rarely occurs with larger atoms (or indeed with the sodium example given above), it is theoretically possible to remove all of the electrons from any given atom, leading to third, fourth, fifth, etc. ionization energies for atoms with large numbers of electrons.
Figure 4: Using the element hydrogen, examples of a cation and anion.
image ©JkwchuiOnce an electron (or electrons) has been ejected from an atom in this manner, it can be accepted by other atoms, and as such, electrons can be transferred from one atom to another. Just as releasing electrons unbalances the charge, accepting electrons causes atoms to become unbalanced in terms of their charge as well, and once again an ion is formed. This time, the ion has a negative charge (a greater number of electrons than protons), and the species is called an anion. (A hydrogen cation and anion are shown in Figure 4.) For example, a neutral chlorine atom, with equal numbers of protons and electrons, can accept an electron from an external source to form a negatively charged chloride anion (Equation 2).
Ions have wildly different properties when compared to their parent atoms, and this transfer of electrons from one atom to another is an example of how a small change in the structure of an atom can make a large difference in the behavior and nature of the particles. For example, sodium metal is unstable, reacting violently with water and corroding instantaneously in air. Thus, coming into contact with free sodium metal would be extremely dangerous. Similarly, chlorine exists as a gas under ambient conditions and it is highly poisonous, scarring the lungs of anyone who breathes it (in fact, free chlorine gas was used as a chemical weapon in World War I). However, when the two substances react with one another, sodium loses an electron forming a cation, and chlorine accepts the same electron to form an anion. The two resulting ions then bond together as a result of their charges, and together create a very common substance – table salt, which is neither reactive nor poisonous.
Comprehension Checkpoint
Bohr ties the unexpected observations to ions
In Bohr’s work, the ionization of one particular element, helium, proved to be the key to unlock the explanation for the unexpected lines that he observed in hydrogen’s spectrum. When a helium atom, which has two electrons, loses an electron to form the helium ion, He+, its electronic structure mimics that of atomic hydrogen, since both species only have only one electron – the helium ion is said to be isoelectronic with the hydrogen atom. However, the helium ion possesses a nucleus with double the charge of a hydrogen atom (two protons as opposed to one proton). Bohr realized this, and suggested that the greater attraction between the electron and He nucleus accounted for the spectral lines that were previously unexplained – the charge of the nucleus affected the energy associated with transfers of electrons between the orbitals. Bohr’s theory was proven correct when spectra were generated using ionized helium that had been purged of hydrogen.
Discovery of a third atomic particle: The neutron
At the close of the 19th century, two different particles were known to exist in the atom, and both possessed an electrical charge – the very small and negatively charged electron and the much larger and positively charged proton. However, by the beginning of the 20th century, evidence began to mount that this was not a complete picture of the atom. Specifically, the mass of protons and electrons in an atom did not appear sufficient to justify the mass of the whole atom, and certain types of nuclear decay suggested that something else might be going on in the nucleus.In 1932, James Chadwick, a British physicist who had studied with, and was working for, Ernest Rutherford at the time, set out to solve the problem. Rutherford had proposed the idea of a neutral atomic particle that had mass as early as 1920, but he never managed to gain traction in the hunt for this mysterious particle.
In 1932 Chadwick further developed an experiment that had been first performed by Frederic Joliot-Curie and Irene Joliot-Curie. They found that by using polonium as a source of alpha particles, they could cause beryllium to emit radiation that, in turn, could be used to knock protons out of a piece of paraffin wax. The Joliot-Curies proposed that this radiation was gamma radiation, a packet of energy with no true mass. As an accomplished researcher of gamma rays and of the nucleus of the atom, Chadwick realized what others had not – that protons were too massive to be ejected from paraffin by mass-less gamma rays. By more carefully measuring the impact of the mystery particle on the paraffin wax and combining this with other measurements, Chadwick concluded that the particles being emitted were not gamma radiation, but a relatively heavy particle that had no charge – a particle named the neutron (Figure 5).
Figure 5: Artistic model of an atom showing the nucleus, with protons and neutrons, and orbiting electrons.
image ©VisionlearningChadwick wrote a paper about his discovery entitled “The Possible Existence of a Neutron,” and it was published in the journal Nature (Chadwick, 1932). In 1935, he was awarded the Nobel Prize in Physics for his discovery. The Joliot-Curies did not go without recognition either. Their work on radioactivity and radioactive isotopes won them the Nobel Prize for Chemistry, also in 1935.
Chadwick’s discovery marked the genesis of induced nuclear reactions, where the neutron is accelerated and crashed into the nuclei of other elements, generating massive amounts of energy (the neutron can easily do this since being neutral it is not repelled from the nucleus in the way that positively charged particles would be). These reactions had a massive impact upon the world as a whole since they spawned thoughts of the atomic bomb and of nuclear energy.
Comprehension Checkpoint
Other changes in the structure of atoms: Isotopes
The neutron also explains the existence of atoms of the same element that have different atomic masses. Isotopes are atoms of the same element (i.e., they have the same numbers of protons) but that differ in the number of neutrons they possess. As a result, different isotopes have similar chemical properties, but their masses, and in some cases their physical behavior, differ. Isotopes are differentiated by their atomic mass, which can be indicated by writing the element’s symbol, followed by a dash and then the mass, or, more commonly, by writing the mass as a superscript before the element symbol. For example, carbon-12 (C-12, or 12C) and carbon-14 (C-14, or 14C) are both naturally occurring isotopes of carbon. Carbon-12 is a stable isotope that accounts for almost 99% of naturally occurring carbon. Carbon-14 is a radioactive isotope that accounts for only about 1 x 10-10 % of naturally occurring carbon, but because it decays to nitrogen with a half-life of approximately 5,730 years, it can be used to date some carbon-containing objects. (Three isotopes of carbon are depicted in Figure 6.)
Figure 6: Carbon isotopes. Each have the same number of protons, but different numbers of neutrons.
There is often more than one naturally occurring isotope of any given, individual element. As a result, the atomic masses that are given on a modern periodic table are the weighted masses of all the known isotopes of each element. For example, naturally occurring chlorine has two principal isotopes, one with 18 neutrons (mass of 35), and one with 20 neutrons (mass of 37). The lighter isotope 35Cl, accounts for almost 76% of its natural abundance, while the 37Cl isotope accounts for only about 24%. Thus the weighted mean is the average mass of naturally occurring chlorine atoms weighted by their relative abundance, or 35.45.
Comprehension Checkpoint
Bohr’s work provided a bridge between several disparate ideas that may never have been linked together without his intervention. He changed the paradigm from applying classical (particle) physics to the model of the atom, to thinking about the application of quantum theory and waves – a truly crucial development in the grand scheme of atomic theory and one that laid the groundwork for future scientists to build upon.
Having traveled from the earliest work on atomic theory through the crucial early part of the 20th century, we still have some way to go in the story of the atom.
The advancements described in this module were grounded in Rutherford’s work, modified by Planck’s insights, and pieced together by Bohr’s genius. Still, there would be at least another decade that had to pass before work by Pauli, Heisenberg, and ultimately Schrödinger led to the full development of modern quantum mechanics that completely describes the atom as we know it today.
This module is an updated version of our previous content, to see the older module please go to this link.