Discovery and Structure of Cells: Cell theory, prokaryotes, and eukaryotes
by Heather MacNeill Falconer, M.A./M.S., Nathan H Lents, Ph.D.
Listen to this reading
00:00Did you know that the human body consists of trillions of individual cells and 200 distinct types of cells? Human cells range in size from 1/12,000 of an inch (a few micrometers) to over 39 inches (more than a meter) long. All living things are made of cells, but in spite of vast differences in size, shape, and function, these building blocks of life share remarkable similarities.
Cells are the basic structural and functional unit of all living things and contain inheritable genetic material.
The activity of a cell is carried out by the sub-cellular structures it possesses.
Cells possess an outer boundary layer, called a cell membrane, cytoplasm, which contains organelles, and genetic material.
There is considerable variety among living cells, including the function of membranes and subcellular structures, and the different types of functions the cells carry out, such as chemical transport, support, and other functions.
There are many discoveries that have changed the course of science and the world. Nikola Tesla’s discovery of alternating currents, for example, helped pave the way for widespread access to electricity, and Louis Pasteur’s discovery that heat and disinfectant could kill bacteria improved food safety and saved millions of lives. In 1655, the English scientist Robert Hooke made an observation that would change the study of biology forever. While examining a thin, dried section of cork tree with a crude light microscope, Hooke observed that he could plainly see the cork to be made up of tiny spaces surrounded by walls, much like a honeycomb, but that the spaces were irregular and shallow (Figure 1). Further, Hooke noted that these "little Boxes" were so numerous that there were "in a square Inch above a Million... and in a Cubick Inch, above twelve hundred Millions [sic]" (Hooke, 1655).
In his landmark book Micrographia, Hooke called these spaces "cells" because they resembled the small rooms monks lived in (cella in Latin). What Hooke’s samples were not able to reveal at the time, though, was that cells are not in fact empty. Though he was diligent in looking at his samples through different magnifications and with various light sources and angles, there were two major obstacles that stood in Hooke’s way of discovering subcellular structures. The first was that the microscope he was using at the time was still too low of a magnification to show that much was contained within the walls of the cells. The second: His samples were of cork – composed of long-dead cells, absent of any cytosol or organelles.
Antony van Leeuwenhoek improves microscopy
In the years immediately following, other scientists would build on the work of Hooke, including Antony van Leeuwenhoek (1632 – 1723), a cloth merchant in Delft, Nederland. Van Leeuwenhoek was not a scientist by formal training, but he was an industrious and curious individual who took great joy in observing the world around him (Anderson, 2009). While working in his haberdashery in the 1670s, van Leeuwenhoek began to experiment with glass-blowing and the construction of microscopes (Figure 2). Using the designs described by Hooke in Micrographia, van Leeuwenhoek built his own microscopes by hand, fabricating every element from the highly-refined lens to the screws used to hold the instrument together (Anderson, 2009).
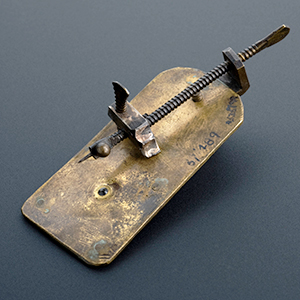
During his lifetime, van Leeuwenhoek constructed hundreds of microscopes and lenses by hand, each one unique. It was with these microscopes and improved lenses that he began to study the world around him and share these observations with institutions like the English Royal Society. One of his first important observations came in August 1674, when he looked at water samples from Berkelse Meer, a lake two miles outside of Delft. In a letter to Henry Oldenburg that September, and published in Philosophical Transactions of the Royal Society, van Leeuwenhoek noted:
I took up some of it [the water] in a Glass-vessel which having viewed the next day, I found moving in it several Earthy particles, and some green streaks, spirally ranged, ... among all of which there crawled abundance of little animals some of which were roundish; those that were somewhat bigger than others were of an Oval figure: On these latter I saw two legs near the head and two little fins on the other end of their body…. The motion of most of them in the water was so swift, and so various, upwards, downwards, and round about, that I confess I could not but wonder at it. I judge, that some of these little creatures were above a thousand times smaller than the smallest ones, which I have hitherto seen.
What van Leeuwenhoek was seeing, we can now presume, were some of the smallest forms of life: protozoa, rotifers, ciliates, and phytoplankton. Van Leeuwenhoek’s descriptions are among the first to identify the unique features of these different microscopic organisms and was the beginning of the discipline we now call microbiology – the study of microscopic organisms.
Comprehension Checkpoint
In the years immediately following van Leeuwenhoek’s discovery of microorganisms in the Berkelse Meer water, his studies unearthed some very important cellular distinctions. Among them was the discovery of single-celled organisms (Figure 3) and structures existing within the walls of Hooke’s originally-thought-empty plant cells (large organelles called vacuoles).
The beginnings of cell theory
Science is not a solitary endeavor, and many important “Aha!” moments have been the result of being in the right place at the right time. Such was the case with the development of cell theory. During a dinner conversation in 1838, German botanist Matthias Jacob Schleiden mentioned to the German physiologist Theodore Schwann that he believed all plants, and plant parts, were composed of cells. Schwann had come to a similar conclusion about animals through his own research and immediately saw the value in sharing such an idea publicly in the scientific sector (and sharing it quickly, before someone else got credit for it). In 1839, his work Microscopic Investigations on the Accordance in the Structure and Growth of Plants and Animals was published and, without giving any credit to Schleiden, formally made the first statement in cell theory: All living things are composed of cells and cell products.
This simple statement was another important turning point in biology. At the time of the book’s publication, Schwann was working in the laboratory of Johannes Peter Müller at the University of Berlin. In a true symbiotic relationship, Müller used his leverage as the University’s Chair of the Anatomy and Physiology Department to promote the text; Schwann continued to work in the laboratory and helped solidify Müller’s lab as the research center of cell theory.
The belief that all living things are composed of cells was soon widely accepted in the scientific community, but how these cells came into being was still an area of unknowing. In the heavily religious atmosphere of the 18th and 19th centuries, any suggestion that life was the result of something other than a Divine Creation was met with great resistance. So most scientists of the time subscribed to the Theory of Spontaneous Generation while they continued to research the structure and function of life (see our Experimentation in Scientific Research module).
As the quality and magnification ability of microscopes continued to improve, the discoveries about the diversity of cells and cell structures also increased. It wasn't long before it was clear that there were two major types of cells – those that are an organism unto themselves (unicellular), and those that are most often part of a larger body (multicellular). Further, within these two types it was noticed that some had a membrane-bound nucleus, and some did not. These two major types were given the names prokaryote (meaning, "before the nut" or "kernel") and eukaryote (meaning, "with the nut" or "kernel"). The "nut" or "kernel" refers to the nucleus (Figure 4).
Comprehension Checkpoint
Prokaryotes and eukaryotes: The case for a shared ancestry
While all life is made up of cells, not all cells have the same structure. In the organization of living things, organisms fall into one of two groups: prokaryotes and eukaryotes. Prokaryotes (archaea and bacteria) and eukaryotes (fungi, plants, animals, and protists) have many defining factors that are different from one another, but their similarities are very important and form the foundation on which a theory of shared ancestry is built.
All prokaryotes and eukaryotes consist of cytosol with ribosomes suspended in it, the genetic material of DNA and RNA, and are enclosed in a membrane. These common elements are chemically and structurally almost indistinguishable. The plasma membrane consists of a phospholipid bilayer, which is a fatty film that surrounds the cell (see our Membranes I: Introduction to Biological Membranes module to learn more). This membrane contains several structures that allow the cell to perform necessary tasks, including pumps and channels that allow substances to move into and out of the cell, and receptors that allow the cell to sense what is in its surroundings and be recognized by other cells (see our Membranes II: Passive and Active Transporters module). This plasma membrane forms a semi-permeable barrier that keeps the cell’s cytosol from leaking out and the surrounding environment from leaking in.
Cytosol is a gel-like fluid consisting of water packed with dissolved nutrients, wastes, ions, proteins, enzymes, and many other molecules. Many chemical reactions take place in the cytosol and it contains particles and filaments that provide shape to cells. Suspended within the cytosol are ribosomes – large molecular machines responsible for translating the information contained in RNA into proteins. The number of ribosomes in a cell depends largely on the cell’s function. (See Figures 5 and 6 for illustrations of the cell structures.)
Both eukaryotes and prokaryotes also have genetic material (DNA and RNA), which carries the instructions for the production of proteins (see our DNA module series). However, likely the most important distinction between the two taxa of cells is that the genetic material of eukaryotes is enclosed within a double membrane, creating a nucleus. Prokaryotes have no such membrane-bound nucleus; their genetic material exists in a nucleoid – an irregularly shaped region within the cytosol.
Although a bacterium seems much different than a mold, and a tree seems very different from a human, inside the cells of all these organisms many things are very much the same. This argues that all living things on Earth are related and descended from a common ancestor. This is called the Theory of Universal Common Descent. Consider the following:
- All living things use DNA for their genetic material. Hypothetically speaking, there are dozens of molecules that could function as a repository of genetic information. In fact, proteins and sugars might have been "better" choices than DNA, since they would allow much more information to be stored in the same size molecule. However, every living cell stores their genetic information in the form of chromosomes made of DNA. In addition, all living things use the same four nucleotides as the building blocks of DNA. Nucleotides could be built in an almost infinite number of ways, but only four are used by life on Earth (Figure 6).
- The genetic code is universal. Not only do all living things store their genetic information using the same molecule, the code for reading the information is identical as well. For example, in a given DNA sequence, Cytosine-Thymine-Cytosine (CTC) codes for the amino acid leucine. This is true in every living cell from bacteria to humans. There is no reason why this would have to be true. The genetic code is like Morse Code: it is purely arbitrary. Any number and combination of DNA nucleotides could serve as a code for any given amino acid. And yet, all life uses the exact same code. (There are a couple of exceptions, but these are very rare.) This common feature of life is what allows us to insert genes from one species into another and have those genes still work properly. For example, it would be extremely expensive to harvest insulin from human donors in order to treat patients with diabetes. Instead, scientists have engineered bacteria that contain the human insulin gene. The gene is read and interpreted the same way in both cells, so the bacteria build a perfectly functional human insulin molecule.
- All cells convert chemical energy in similar ways. The energy that reaches the planet from the sun could be harvested in an almost infinite number of ways. However, the process and enzymes for photosynthesis are strikingly similar among all photosynthetic cells, from cyanobacteria and plankton to oak trees and lily pads. Similarly, all cells consume macromolecules and convert their energy in astonishingly similar ways. The enzymes of glycolysis, the process of breaking down glucose, are shared among all living cells. In addition, all cells make and use ATP molecules as their general “currency” for transferring energy in its many chemical reactions. There are literally thousands of molecules that could be used for this purpose, including many that would function more efficiently than ATP. The chemical reactions of energy conversion are remarkably similar in all cells on Earth.
- All ribosomes are structurally and functionally similar. Structures called ribosomes are responsible for interpreting the genetic code of DNA, received in the form of mRNA, and building proteins according to that code. The ribosomes of all prokaryotes are almost exactly the same, and so are the ribosomes of all eukaryotes. Between eukaryotes and prokaryotes, although there are differences, the overall structure is remarkably similar. All ribosomes have two parts: a big subunit and a small subunit. They operate in nearly identical mechanisms.
- All biological membranes are similar. From the plasma membrane of bacteria to the nuclear envelope of animals, the water-tight membranes that establish separate compartments within and around a living cell are extremely similar. On the one hand, this is no surprise because the properties of phospholipids are quite unique. On the other hand, starting with very basic chemical building blocks, like those found on the early Earth, many possible membrane-forming molecules could have emerged. In fact, scientists can now synthesize much better, simpler, and more stable membranes. That all life uses the same basic membrane structure is strong evidence that once membranes first evolved, they were passed to descendants with little changing along the way.
These are but a few of the pieces of evidence for the Theory of Universal Common Descent.
Prokaryotes have been documented in the fossil record as far back as 4.2 billion years (see Figure 8). Eukaryotes, however, can only be documented as far back as 2.7 billion years, leaving 1.5 billion years of evolution to take place between. For this reason, scientists believe that eukaryotes evolved from prokaryotes long after the central features of living cells had already emerged.
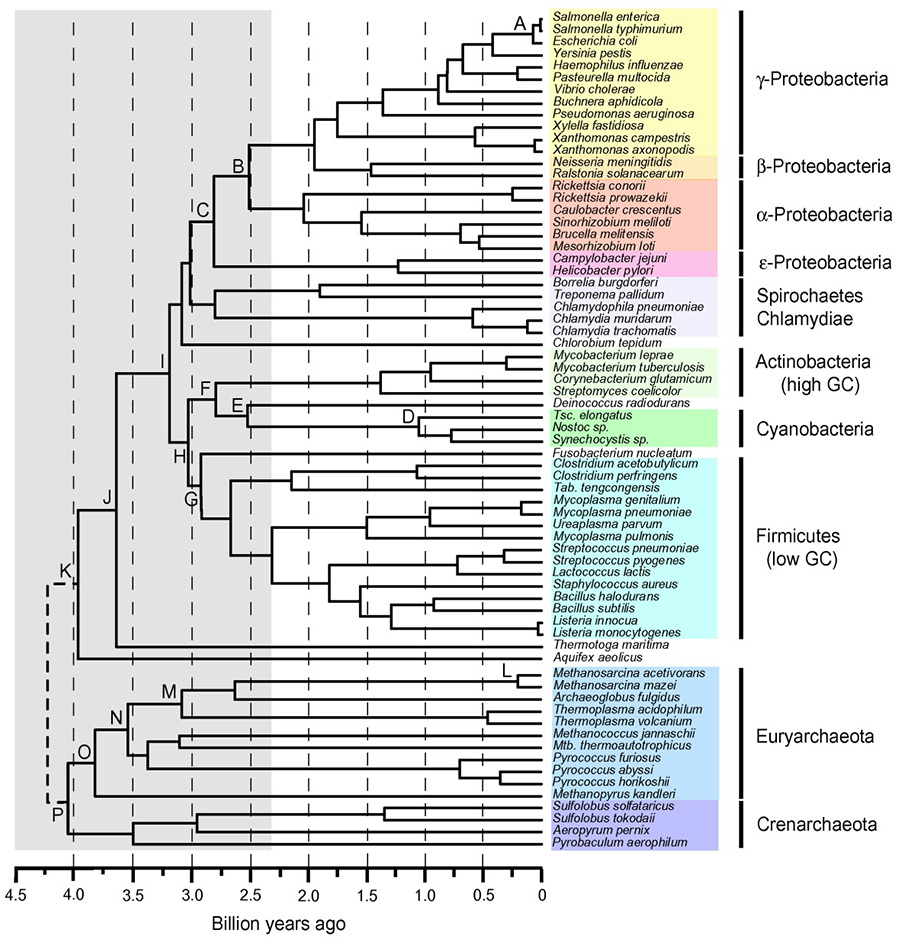
The defining feature of eukaryotes, the nucleus, first evolved as an in-folding of the plasma membrane, which formed a compartment to house and protect the DNA. This in-folding evolved into the nuclear envelope – the double membrane of the nucleus (Figure 9). In addition, as discussed in our Cellular Organelles I: Membrane-bound Organelles module, the Theory of Endosymbiosis suggests that a small aerobic bacteria was able to penetrate the plasma membrane of a larger, anaerobic prokaryote, probably an archeon, and survive, living symbiotically with the host cell. Over time, the small aerobic bacteria evolved into the organelle we know today as the mitochondrion.
Comprehension Checkpoint
Differences between cell types: Organelles and their functions
All basic chemical and physiological functions – repair, growth, movement, immunity, communication, digestion – are carried out inside of cells, and the activities of cells depends on the activities of the structures within the cell (including the organelles). This means cells can convert energy from one form (which, depending on the cell type, can be in the form of light, sugar, or other compounds) into another. For example, cells can digest the building blocks of other organisms that it has eaten and used the released energy to build its own materials such as proteins, carbohydrates, and fats.
Most of the activities of a cell are carried out via the production of proteins. Proteins are large molecules that are made by specific organelles within the cell using the instructions contained within its genetic material (see our series on DNA: DNA I: The Genetic Material, DNA II: The Structure of DNA, DNA III: The Replication of DNA). Depending on the type of organism, specific organelles may or may not be present in a cell.
In addition to the plasma (cell) membrane, cytosol, ribosomes, and nucleus, the typical components of eukaryotic cells include: mitochondria, transport vesicles, endoplasmic reticulum, Golgi bodies, and lysosomes. In addition to these, photosynthetic (plant) cells will have a cell wall, chloroplasts, and a central vacuole.
Prokaryotic cells, however, do not contain any membrane-bound organelles. Instead, they can include plasmids, a cell wall, and in the case of photosynthetic prokaryotes, thylakoids. Table 1, below, lists the function of each type of organelle and which group of cells it is found in.
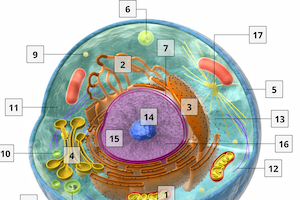
Interactive Animation: The Structure of Animal Cells
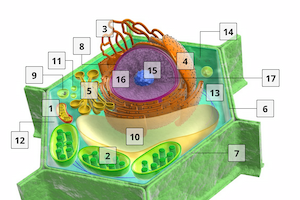
Interactive Animation: The Structure of Plant Cells
Membrane-bound Organelles | |
---|---|
Independently replicating | |
![]() |
Mitochondrion (plural: mitochondria) The "power supplier" for the cell, generating most of the ATP used in cell processes through the conversion of nutrients into energy. Also involved in cell signaling, controlling the cell cycle and cell growth, and cellular differentiation. Found in all eukaryotes. |
![]() |
Chloroplasts A chlorophyll-containing plastid responsible for converting sunlight and carbon dioxide into oxygen and sugar. Found in plants and algae. |
Endomembrane System | |
![]() |
Smooth Endoplasmic Reticulum A series of sac-like membranes responsible for the synthesis and storage of lipids, phospholipids, and steroids, as well as the metabolism of carbohydrates. |
![]() |
Rough Endoplasmic Reticulum A series of sac-like membranes studded with ribosomes, responsible for the synthesis and export of proteins |
![]() |
Golgi Apparatus Functioning like a distribution center, the Golgi Apparatus gathers simple molecules and creates more complex molecules. Once created, those complex molecules are transported to other organelles, stored in vesicles, or exported from the cell. |
![]() |
Plasma Membrane A layer of phospholipids and proteins that forms a barrier between the inside of the cell and the outside environment. |
![]() |
Cell Wall Found in plants, fungi, and some protists, a structure outside of the plasma membrane that provides strength, support, and protection. |
![]() |
Lysosomes A specialized compartment containing hydrolytic enzymes. The role of lysosomes is to digest sugars, proteins, and other "foodsâ a cell absorbs. |
![]() |
Nuclear Envelope A double-membrane surrounding the nucleus. This membrane provides a barrier between the nucleus and the cytosol. |
![]() |
Peroxisome Similar to lysosomes, these contain enzymes used in a variety of reactions, including oxidation reactions. In plant seeds, peroxisomes convert stored fatty acids to carbohydrates, providing energy for germination. In plant leaves, they are involved in photorespiration. |
![]() |
Vacuoles A compartment used for storage of nutrients, water, and waste. In plants, the central vacuole plays an important role in providing structure. |
![]() |
Vesicles A small spherical compartment composed of a lipid bilayer and internal fluid used to exchange cargo between organelles of the endomembrane system. Specialized vesicles play a variety of roles. |
![]() |
Cytosol Otherwise known as intracellular fluid (ICF), the liquid matrix found within a cell that holds other organelles and allows intracellular processes to take place. |
Non-membrane-bound Organelles | |
---|---|
![]() |
Cytoskeleton A complex network of protein fibers that give the cell its shape. These fibers also play important roles in assisting vesicles and organelles to move around the cell, as well as the movement of chromosomes during cell division. |
![]() |
Ribosome The protein builders of the cell. Ribosomes are responsible for constructing amino acids to build amino acid chains. |
![]() |
Nucleolus Where ribosomes are made inside the nucleus. |
Comprehension Checkpoint
Cell theory expands
In 1855 German biologist Rudolf Virchow realized that the widely held idea that organisms spontaneously generate out of non-living matter did not make sense, and an idea proposed by Polish-German embryologist Robert Remak might be correct. Remak, a friend and colleague of Virchow, had put forth the idea that cells generate from preexisting cells, and not from things like dust and dead fish. Plagiarizing Remak’s idea, Virchow officially added to cell theory in 1858 with the statement: Every cell originates from another existing cell like it. This statement, along with Schwann's declaration that "All living things are made of cells," forms the basis of modern cell theory.
While it is true that all cells are produced by the division of preexisting cells (in other words, through reproduction), we now know that the process of how cell reproduction takes place differs between prokaryotes and eukaryotes – and that the speed at which it takes place within those two groups also differs.
Prokaryotes replicate through binary fission, a process by which a single prokaryote cell simply divides itself in half. Because prokaryotes are single-celled organisms, every time a prokaryotic cell divides it is reproducing. Prior to the fission, the genetic material (DNA) is copied within the cell; then the two molecules of DNA attach to opposite sides of the membrane. The membrane then grows between the two molecules, forming a separation. When the prokaryote has doubled in size, the cell begins to pinch inward and a cell wall forms, dividing the cell in half (see animation).
Eukaryotes, however, reproduce through a more complicated process involving several phases, including interphase, mitosis, and cytokinesis (see our Cell Division I: The Cell Cycle module). During the first half of interphase, called the G1 phase, cells take in nutrients and nearly double in size. Then, in S-phase, the DNA inside the cell’s nucleus replicates, making a complete copy of itself. Following this is the G2 phase, where the cell checks and corrects any errors that may have occurred in that DNA replication and grows a little bit more. If all has gone well, the cell proceeds to mitosis, or M phase, when the two DNA copies are separated and the nucleus splits to create two identical daughter nuclei. Finally, the rest of the cell splits in two, each with its own new nucleus, in a process called cytokinesis.
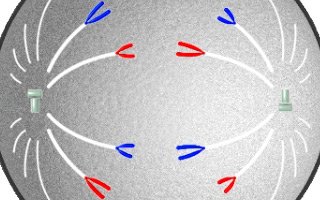
Interactive Animation: Prokaryote vs. Eukaryote Cell Division
All cells contain genetic material in the form of DNA that is passed from the parent cell to the two daughter cells. However, in prokaryotes that genetic material is in a circular form, while in eukaryotes the genetic material is in linear strands. For prokaryotes, binary fission is a means of reproducing and increasing numbers of the population. For example, Salmonella bacteria can grow so fast that the population doubles every thirty minutes. This means that a single Salmonella bacterium sitting on a tiny piece of raw chicken left in the kitchen sink after dinner can give rise to a million descendants by the next morning.
However, most eukaryotes are multicellular. Most of the cell division that takes place is not to produce more organisms, but rather to allow the organism to grow and develop and to repair and renew tissues. Nevertheless, each and every cell division follows the same complex pattern. Even the most rapidly dividing human cells take more than twenty hours to a complete a single division cycle (with only very rare exceptions). In addition, it is not just the DNA and the cytosol that must be duplicated and split between the two daughter cells: Eukaryotic cells contain many specialized organelles suspended in their cytosol. During cell division, the many organelles must expand, split up, and be distributed more or less evenly between the two daughter cells. Unlike the other eukaryotic organelles, mitochondria and chloroplasts contain their own unique genetic material. They replicate themselves independently when more are needed and are then passively distributed to the two daughter cells during cytokinesis.
Comprehension Checkpoint
Cell variety within organisms
Cell diversity extends beyond the differences between prokaryotes and eukaryotes, and between the different kingdoms of organisms (plants, animals, etc.). There are also major differences in cells within an individual organism, reflecting the different functions cells perform. For example, the human body consists of trillions of cells, including some 200 different cell types that vary greatly in size, shape, and function. The smallest human cells, sperm cells, are a few micrometers wide (1/12,000 of an inch), whereas the longest cells, the neurons that run from the tip of the big toe to the spinal cord, are over a meter long in an average adult.
Human cells also vary significantly in structure and function. For example, only muscle cells contain myofilaments – protein-containing structures that allow the cells to contract (shorten) and, as a result, cause movement. The eye contains specialized cells called photoreceptors that have the ability to detect light. These cells contain special chemicals called pigments that can absorb light and special structures that release chemicals onto other cells which can then send electrochemical currents to the brain, a process we perceive as vision.
Plants also contain a wide variety of cell types. There are specialized cells called collenchyma that provide structure without restricting growth and flexibility. These cells lack secondary cell walls, and their primary cell walls lack a hardening agent, which especially helps young plants grow quickly and be resilient to wind and water. Other types of plant cells include xylem, whose purpose is to transport water throughout the plant, and phloem, whose purpose is to transport organic nutrients.
The realm of cellular discovery is one that is still alive and well, despite its extensive history. In 2013, a group of European scientists identified a new organelle inside the cells of tannin-producing plants, like grapevines and tea trees (Brillouet et al., 2013). Called tannosomes, the organelles originate within the chloroplasts and are responsible for creating the bitter tasting polyphenol that wards off predators and gives wine and tea their familiar “dry” feeling in the mouth. And in the same year, researchers in the United States identified that the types of proteins developed by ribosomes occurred in phases along with the phases of the cell cycle (Stumpf et al., 2013). Identifying which proteins are produced when has implications for cancer research, since hypotheses currently exist suggesting inefficient protein synthesis (translation) in cancer cells. While it is easy to think that modern technological advances means that we’ve discovered all the components of cells, we must remember that, like Robert Hooke, there are sometimes things preventing us from seeing everything and that new discoveries may still await.
This module is an updated version of The Discovery and Structure of Cells.
Table of Contents
Activate glossary term highlighting to easily identify key terms within the module. Once highlighted, you can click on these terms to view their definitions.
Activate NGSS annotations to easily identify NGSS standards within the module. Once highlighted, you can click on them to view these standards.